Therapeutic potential of tumor associated macrophages
Introduction
Advances in the understanding of oncology have shifted the paradigms from tumor-cell-centric theory to the tumor microenvironment concept. Indeed, early seminal work elegantly linked inflammation to neoplasms and the cancer-related inflammation (CRI) can arise before tumorigenesis or be driven by local cancer cells (1). Accordingly, tumor-promoting inflammation and evasion of anticancer immune destruction are considered as hallmarks of cancer (2). Herein, tumor-associated macrophages (TAMs) are the most abundant infiltrates and indispensable accomplices during neoplastic progression (3).
Macrophages are a key component of the innate immune system, which phagocytose microbes and present antigens during acute infection (M1-like phenotype), while promoting wound healing and cell proliferation in the late stages (M2-like phenotype) (4). In the tumor microenvironment, monocytes and monocytic-myeloid derived suppressive cells (M-MDSCs) are recruited from the circulation and, like tissue resident macrophages, primed to an M2-like TAM phenotype (5). Local accumulation of aberrant metabolites and a variety of cytokines and growth factors derived from tumor cells or immune cells are indicated in the polarization of intratumoral macrophages through distinct signalings, such as JAK-STAT6 and PI3Kγ pathway (6). The modulating factors and the pivotal molecules in the pathways serve as attractive therapeutic targets of tumor controlling interventions.
In the context of tumor, TAMs have an essential role in cancer development, which support the survival and proliferation of neoplastic cells, circumvent antitumor immune responses, facilitate angiogenesis and metastasis, and affect the efficacy of various regimens. In this review, we focus on the acquisition of tumor cells of stem-cell properties, the infiltrative growth of vascular network, the organotropism of metastasis and the development of resistance to endocrine therapy, which are incompletely understood and attractive field regarding TAMs. Therapeutic interventions targeting these functions of TAMs have shown preliminary promise in the clinical arena, accompanied by a series of challenges and opportunities.
TAMs and epithelial-to-mesenchymal transition (EMT)
Among the highly mutant and heterogeneous tumor cells, the subpopulation of cancer stem cells (CSCs) is endowed with self-newel and differentiating capacity, enhanced mobility and aggressiveness, and insensitivity to therapeutic applications. EMT is critical to induce stem cell-like properties in cancer cells, with the loss of epithelial traits and intercellular adherence and acquisition of mesenchymal characteristics and intensive migratory capacity (7,8). At the invasive front of mammary carcinomas, mesenchymal-like tumor cells program macrophages to a TAM-like population via GM-CSF secretion. Lactate accumulation in the surrounding microenvironment abolishes the antitumor potential of GM-CSF in supporting M1 macrophages. Reciprocally, the chemokine CCL18 synthesized by TAMs is fundamental in EMT and GM-CSF synthesis of mammary cancer cells in co-culture studies and humanized mice (9). As a receptor of CCL18, PITPNM3 on the surface of tumor cells provokes NF-κB signaling via Pyk2/Src pathway, which mediates the stabilization and nuclear accumulation of Snail, a crucial transcription factor for EMT (10,11). NF-κB activation in mammary tumor cells raises Lin28b level and downregulates miR98 and miR27b, which reinforce the invasion and lung metastasis of neoplasms (12). Increased GSK-3β activity in response to PI3K/Akt pathway activation is also involved in CCL18-induced Snail upregulation and maintenance (13). High level of GM-CSF or CCL18 shows noteworthy correlation with EMT, metastasis, and poor survival in patients with breast cancer (9). Treatment of anti-GM-CSF or anti-CCL18 antibodies or inhibition of lactate production breaks the GM-CSF-CCL18 loop and tempers mesenchymal traits of cancer cells (9,11). Currently, the utility of drugs targeting GM-CSF-CCL18 loop is attracting further estimation in the clinical arena.
Activation of TGF-β signaling presents a principal strategy for macrophage-dependent mesenchymal and stem-cell conversion (8). In breast cancer, TGFβ-Smad pathway collaborates with Wnt-β catenin signaling to precipitate initiation of the EMT program and generates autocrine loops to perpetuate mesenchymal state (14). TGF-β-induced CELF1 protein binds to the conserved cis-element in 3’-UTRs of a cohort of mRNAs encoding EMT drivers and raises their translation. Elevated expression of CELF1 is observed in human breast cancer tissue and associated with accelerated metastasis (15). Activation of the Ras effector Blimp-1 in response to TGF-β1 resulted in a decline of BMP-5 and subsequent upregulation of Snail, prompting EMT process and tumor invasion (16). Snail not only amplifies vimentin production and cell aggressiveness while depriving E-cadherin expression (17), but bridges p53 and histone deacetylase1 (HDAC1) and facilitates the proteasomal degradation of p53 (18). TGFβ–induced EMT involves two sequential jumps from epithelial to partial EMT and then to mesenchymal phenotype. Herein, the double-negative feedback control between Snail and miR-34, ZEB1 and miR-200 undergo stepwise activation (17). Therapeutic agents targeting TGF-β signaling have already been developed and estimated in both preclinical and clinical testing (19,20). However, this cytokine has critical roles in a myriad of physiological processes involving immune and stromal cells, making it difficult to specifically target tumor EMT (7). Furthermore, TGF-β signaling exerts an anti-proliferative effect in the initiation stage of tumorigenesis (21). Inhibition of this pathway facilitates the development of various types of human cancers (22), suggesting for appropriate stratification of the patients in the administration of this kind of drugs.
Additional signaling pathways and molecules involved in the tumor cell EMT are considered potential therapeutic intervention target to reverse tumor stemness and repress tumor mobility. Mesenchymal cancer cells exhibit high expression of EphA4, which mediates TAMs-triggered production of robust cytokines like IL-6, IL-8, GM-CSF and maintenance of cancer cells in the stem-cell state (23). The extracellular matrix (ECM) contributes to the mesenchymal conversion of high-grade tumor cells through its modifying activity of the immune microenvironment and myeloid suppressor cells. Interrupting the crosstalk between cancer cells and MDSCs with aminobisphosphonates reverses the EMT/CSC phenotype of tumor cells, rendering them sensitive to chemotherapy agents (24). Metabolic alteration has a significant role not only in the immune evasion of neoplasms but during EMT program and metastasis. At tumor invasive front, the upregulation of NOS2 production of M-MDSCs facilitated tumor aggressiveness, self-renewal and EMT (25). Anti-Ang2 intervention in the neoplasms deficient in PDGFRβ+ pericytes recovers the vascular structural integrity, improves oxygen delivery and attenuates hypoxia-induced EMT in cancer cells (26). Preliminary antitumor activity and tolerability of AMG386, an Ang1/2-Tie2 inhibitor, have been evaluated in clinical trials in adult patients with recurrent advanced solid tumors (27).
Angiogenesis and anti-angiogenic therapy
Pre-malignant epithelial tumors are typically surrounded by a basal lamina which separates the neoplasms from a vascularized adjacent stroma (28). The malignant transition of benign cancers is always accompanied by proliferative vasculature infiltration, providing a steady flow of oxygen and nutrients for tumor outburst (29). Intratumoral macrophages promote the development of new vessels from the pre-existing vasculature, termed angiogenesis, through surface molecule interaction and secretion of bioactive products, which encompass pro-angiogenic growth factors and the matrix proteases (30). As an important source of VEGFA (31), TAMs mediate the engagement of VEGFR2 on endothelial cells (ECs) and the formation of tip cells, which guide the growth of new vascular sprouts (32). Additional macrophage-derived cytokines and growth factors, including placental growth factor (PlGF), fibroblast growth factor 2 (FGF2), VEGFC and tumor necrosis factor (TNF) are indicated in the “angiogenic switch” of tumors (33). TAMs synthesize matrix metalloproteinases (MMPs), cathepsins and plasminogen activator and, through ECM degradation, facilitate the infiltrative development of neo-vessels (34,35). Moreover, these proteases modulate the bioavailability of the pro-angiogenic factors trapped in the perivascular niches (34,35). Genetic or pharmacological abrogation of MMP9 attenuates the vascularization in a mouse model of cervical cancer (36) and in human ovarian cancer xenografts (37). Besides, substantial studies have implicated Tie2-expressing perivascular TAMs in the support of tumor angiogenesis through crosslinking with ECs expressing Ang2, a ligand of Tie2 (38). In a transplant sarcoma model, AKT activation in the macrophages provoked by Tie2 protects them from chemotherapy-induced apoptosis (39). Tie2 inactivation in TAMs at the genetic level impairs their ability to sustain tumor angiogenesis (40). Elimination of broad TAMs by genetic or pharmacological means restrains tumor vascularization in various cancer models (31,41). In human tumors, the abundance of TAMs is frequently correlated with increased vascular density and poor patient outcome (42).
Efforts have been made to suppress tumor angiogenesis and block malignant progression through the reduction in blood supply (30,32). By striking contrast, the pharmacological inhibition of VEGFA elicits acute vascular pruning but no delay or even exacerbation in tumor development in mice and humans (43). This is consistent with the notion that tumors grow neo-vasculature through VEGFA-dependent and independent modes and myeloid cells, in particular TAMs, sustain tumor angiogenesis through various pathways (30). Moreover, anti-angiogenic drugs create an acute hypoxic environment and provoke a surge of TAM influx via chemokines like CSF1 and CXCL12 (33,44). The overshoot of TAMs reverts the anti-angiogenic effect and provides significant support for tumor metastasis. Strategies have been switched to normalize the neo-vasculature to restore pericyte coverage, increase tumor perfusion and mitigate hypoxia (45). Selective impairment of Tie2+ TAMs by blocking Ang2 or Tie2 signaling impedes their association with ECs (40) and induces vascular normalization and reduction (46), posing a promising therapeutic opportunity for antiangiogenic treatment.
The formation of pre-metastatic niches
Increasing number of studies have elucidated that ahead of the arrival of CTCs, the primary tumor selectively and actively primes the “soil” at distant organ sites for their subsequent seeding (47). Tumour-derived factors and exosomes induce the microenvironmental reprogramming of pre-metastatic niches and infiltration of stromal cells, which are essential for vascular alteration and matrix remodeling (48) (Figure 1).
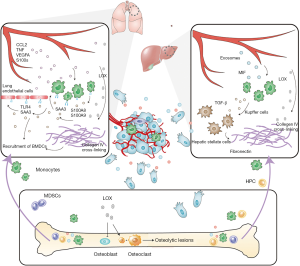
Clinical evidence has unveiled the existence of pre-metastatic niches in sentinel lymph nodes from patients with breast, prostate, colorectal and renal cell carcinomas (49). Cytokines and growth factors secreted by primary tumor, such as TNF, VEGFA and CCL2 travel through the circulation and elicit the production of S100A8 and S100A9 by myeloid and ECs in the pre-metastatic niches (50,51). During this process, S100 proteins also facilitate the secretion of serum amyloid A3 (SAA3), which mediates the activation of NF-κB signaling in pulmonary ECs and macrophages in a TLR4 dependent manner (51). The inflammatory state of these stromal cells accelerates the recruitment of bone marrow derived cells and seeding of primary tumor cells, where MD-2, a co-receptor of TLR4, plays a role (50). For example, in the pre-metastatic lung, MMP9 production of the infiltrating myeloid cells is responsible for abnormal and leaky vasculature alteration before tumor cell arrival (52). Besides, exosomes expressing macrophage migration inhibitory factor (MIF) from pancreatic ductal adenocarcinomas are up-taken by Kupffer cells in the liver, which in turn promote fibronectin production of hepatic stellate cells through their secretion of TGF-β. Local deposition of fibronectin enhances the recruitment of macrophages and the priming of pre-metastatic niches (53). Accordingly, exosomal MIF can be considered an early marker for the development of liver metastasis (53). ECM remodeling and stiffening is pivotal in the preparation of colonizing niche for tumor cells. LOX produced by hypoxic primary tumor cells travels through the bloodstream and accumulates with fibronectin at distant organs, where it mediates the cross-linking of collagen IV and promotes the adhesion of CD11b+ monocytic cells. Attracted CD11b+ cells cleave collagen, reinforce tumor invasiveness and further recruitment of myeloid cells partially through MMP2 synthesis (54). In the skeletal microenvironment, LOX facilitates osteoclast activation and the formation of osteolytic lesions, yielding a platform for tumor cell colonization and bone-tropism. High expression of LOX is associated with skeletal metastasis in ER- breast cancer and identifies patients that will benefit from adjuvant bisphosphonate treatment in reducing bone metastasis (55). In addition, the exposure of macrophages to IL-4 upregulates their miR-142-5p level via STAT6 signaling and downregulates miR-130a-3p through histone deacetylation, which promote the M2 polarization and profibrogenic activities of macrophages (56).
Therapeutic strategies directed against the formation of pre-metastatic niches could impair the establishment of metastasis in an early stage. The inhibition of either TLR4 or SAA3 reduces the mobilization of BMDCs and suppresses lung metastasis in tumor-bearing mice (51). The expression of protein tyrosine phosphatase receptor-type O (PTPRO), a negative regulator of VEGFA, FGFR and PDGF, impedes the “soil-priming” for the seeding of circulating tumor cells in preclinical models of breast cancer (57). LOX depletion with β-amino-propionitrile (βAPN) shows promise in the prevention of myeloid cell recruitment and pre-metastatic niche establishment (54,55). Although remarkable progress has been made in our understanding of the generation and evolution of pre-metastatic niches, further investigations are still in request into the mechanism and translation of the biology to diagnostic and therapeutic approaches.
Colonization and overt metastasis formation
In addition to tumor organotropism, TAMs provide pivotal support for the seeding and outgrowth of tumor cells. Once arriving at pulmonary vasculature, the circulating tumor cells generate microparticles in response to shear flow and interact with resident macrophages. Tumor-ingesting macrophages pile up in the pulmonary interstitium and initiate the colonization of neoplastic cells (58). Notably, the elevation of N-acetyl-galactosaminyltransferase14 (GALNT14) in tumor cells facilitates the secretion of chemokines like CXCL1/2 and the influx of TAMs to the metastatic sites. The modification of FGFR1 on tumor cells by GALNT14 potentiates the proliferative effect of TAM-derived FGF, augmenting macrophage-stimulated continuous tumor growth within the lung microenvironment (59). Myeloid cell infiltration following CXCL1/2 gradient constitutes an instrumental source of the calprotectin S100A8/9, which avails the survival and proliferation of colonizing tumor cells (60). Furthermore, VEGFR1 tyrosine kinase signaling in TAMs regulates CSF1 expression in a p-FAK1 dependent manner, creating an autocrine pathway critical for the persistent growth of the seeded metastatic tumor. Ablation of VEGFR1 attenuates pulmonary colonization of mammary tumor even after initiation of metastatic colonies (61). MiR-21 and miR-29a expression are specifically upregulated in TAMs but not primary tumor by the CSF1-ETS2 signaling pathway. Deletion of these microRNAs making use of Dicer abrogates neovascularization and viability of lung-seeded cancer cells via activation of anti-angiogenic genes (62). Selective inhibition of class IIa HDAC using TMP195 affects the responses of human monocytes to CSF-1 and GM-CSF in vitro (63). In the MMTV-PyMT model, TMP195 treatment promotes vascular normalization and abates mammary pulmonary metastases by modulating the expansion of intratumoral macrophages and their phagocytic activities (64). In addition to EMT, TGF-β from stromal cells like macrophages increases Id1 expression in neoplastic cells through Smad3/Smad4 pathway, which opposes the activity of Twist and leads to mesenchymal to epithelial transition (MET) during pulmonary inhabitation of breast cancer (65). Also, versican secretion of monocytic cells plays pivotal roles in the MET of cancer cells and establishment of macrometastases (66). Beyond modeling the matrix, LOX up-regulates the EGF-like domain-containing protein Matrilin2 through HTRA1 and TGF-β1 signaling, which traps EGFR at tumor cell surface and enhances EGF-mediated tumour growth and progression (67). Tenascin C of tumor cell or stroma origin initiates pulmonary outburst of neoplastic colonization through MSI1-dependent NOTCH signaling and LGR5 elevation in the WNT pathway (68).
In the bone metastatic niches, the expression of c-Src potentiates PI3K-AKT signaling induced by macrophage-derived CXCL12 and protects breast tumor cells against the pro-apoptotic effect of TRAIL, supporting the outgrowth of indolent tumor cells (69). Furthermore, integrin α4β1 expression on monocytic progenitors interacts with VCAM-1-expressing cancer cells and amplifies osteoclast activity through NF-κB pathway in a metastatic dormancy model, facilitating the outgrowth of skeletal metastases (70). TAM-attracting chemokines are attractive therapeutic targets in anti-metastatic treatment and undergoing clinical tests in solid tumors. AMD3100, an FDA-approved small-molecule inhibitor of CXCR4, has shown and preclinical response in ovarian and breast cancer (71,72). Currently, AMD3100 is in widespread clinical investigating in haematological and solid tumors. Besides, CCL2 activated TAMs increase CCL3 secretion and facilitate their retention in an autocrine manner. Genetic deletion of CCR1, a receptor of CCL3, reduces tumor metastasis (73), rendering CCR1 a potential target for anti-metastasis regimen.
TAMs and endocrine therapy resistance
Increasing evidence has shown that TAMs exert an indispensable influence on the antitumor efficiency of cytotoxic chemotherapy, irradiation and immunotherapeutic agents (74). Nevertheless, micro-environmental regulation of tumor response to endocrine therapy is incompletely understood. Since prostate cancer display prominent dependency on the androgen receptor (AR) signaling, castration therapy has been a focus of treatment of prostate cancer (75). Likewise, hormone-responsive breast cancer comprises the vast majority of mammary tumors. Endocrine therapy blocking either estrogen production through aromatase inhibitors or the binding of estrogen to its receptors through tamoxifen or fulvestrant is the current standard-of-care for patients with estrogen receptor (ER) positive tumors (76).
However, the secretion of pro-inflammatory cytokines and growth factors, for example, by TAMs, is associated with the development of tolerance to endocrine therapy through aberrant activation of ER/AR signaling or compensatory excitement of integrated pathways. In patients with prostate cancer, the enrichment of MDSCs in the circulation shows correlation with tumor metastasis (77). In a chimaeric mouse model of metastatic castration-resistant prostate cancer, MDSC diminishing therapy with the use of Cabozantinib and BEZ235 shows a robust antitumor response in combination of immune checkpoint blockade. Herein, the combined therapy impairs the infiltration and immunosuppressing activity of MDSCs through the elevation of IL-1R antagonist, restoring the sensitivity of tumor cells to AR depriving agents (77). In breast cancer, macrophage-derived IL-1β and TNF-α activate IKKβ signaling in tumor cells and mediate phosphorylation of ERα at S305. The cytokine-dependent structural change of ERα facilitates the transcriptional induction of proliferative and anti-apoptotic genes even in the context of estrogen deprivation or tamoxifen treatment (78). TNFα-mediated pro-inflammatory signaling is a significant driver of the redistribution of FoxA1 and NF-κB to new genomic loci, which increases the chromatin accessibility of latent ERα binding sites and gives rise to altered patterns of gene expression in breast cancer (79). Moreover, in response to PGE2, the prostaglandin receptor EP4 activates cAMP signaling and ERα through its cofactor CARM1 in a ligand-independent manner, rendering tumor cells insensitive to endocrine therapy (80).
As the hormone receptor signaling is highly integrated with various pathways, the excessive activation of growth factor receptors like EGFR and FGFR raises their crosstalk with ER-mediated mitogenic signaling and leads to estrogen-independent cell proliferation (76). Tamoxifen-resistant tumor cells display elevated sensitivity to the stimulatory effect of EGF and remarkable dependence on the EGFR/MAPK pathway for persistent growth after durable tamoxifen exposure (81). TAM-derived FGF2 binds to and triggers the phosphorylation and dimerization of FGFR1 on cancer cells, driving downstream kinase cascades like MAPK and PI3K signaling and tolerance to estrogen deprivation (82). Prolonged hyperactivation of MAPK boosts the recruitment of ERK2 and c-Jun to chromatin at the ESR1 gene locus, resulting in the transcriptional repression of the ESR1 and estrogen-independent tumor progression (83). Proteomic profiling of cancer cells under long-term estrogen starvation reveals heightened activation of PI3K/Akt/mTOR pathway, which is associated with poor outcome in patients after adjuvant endocrine therapy (84). Blocking PI3K or mTOR restrains the emergence of estrogen-independent cells and restores tumor response to estrogen-depriving treatment (84). Similarly, BEZ235 and Cabozantinib treatment in castration-refractory prostate cancer induces downregulation of pEGFR and inhibition in PI3K-Akt signaling, leading to a reduction in tumor progression and spread (77). This is in concert with the notion that there is a reciprocal feedback loop between AR signaling and PI3K-Akt pathway (85). However, in PTEN-mutated prostate cancer, PI3K pathway is mainly driven by PI3Kβ. Strikingly, PI3Kβ abrogation in this type of tumor results in a rebound in PI3K signaling through a compensatory upregulation of PI3Kα. Fortunately, the combination of PI3Kα, PI3Kβ and AR blockade potently confines the development of prostate cancer (86). Currently, therapies targeting PI3K pathway are undergoing clinical investigation in castration-resistant prostate cancer (75).
As mentioned above, the acquisition of stem-like phenotype following EMT process endows cancer cells with enhanced aggressiveness and refractoriness to endocrine therapy. It provides a rationale for the investigations to determine the efficiency of targeted therapies against the key cytokines and signaling in EMT in combination with endocrine therapy.
Conclusions
A growing body of studies have provided a framework for microenvironmental regulation in tumor initiation, progression, angiogenesis, immune evasion, metastasis and therapeutic resistance. Thereinto, TAMs serve as pivotal accomplices and an attractive therapeutic target in the suppression of various type of tumors. In the clinical arena, approaches against TAMs have already shown promise over recent years. For example, pharmacological depletion of TAMs by a selective CSF1R inhibitor, PLX3397, induced a durable suppression in tumor growth among patients with tenosynovial giant-cell tumors (87). In pancreatic ductal adenocarcinoma, CCR2 blockade through PF-04136309 elicited image-proven tumor response in 49% patients and local tumor control in 97% patients (88). However, challenges exist in the translation of the TAM-targeting approaches into clinical benefit in patients with neoplasms. Firstly, a large proportion of the studies above employed mouse cancer models for mechanistic exploration of TAMs in the promotion of tumor progression. Interrogation of clinical samples from tumor-bearing patients, instead, will provide significant information of the impact of TAMs or TAM-targeting therapies on tumor cells and the microenvironment, especially in the condition of metastatic malignancies and under therapeutic stress. Next, the remarkable inherent heterogeneity among different tumor models as well as distinct subtypes of each cancer limits the applicability of interventions against TAMs. This suggests for appropriate identification for potential patients that will benefit from microenvironmental treatment alone or in combination. Moreover, resistance to TAM-targeted therapies or even tumor recurrence emerges in several cancer models. CSF-1R blockade through PLX3397 showed limited efficacy in patients with glioblastoma (89). In preclinical trials, long-term CSF-1R inhibition induces a rebound in TAMs partially via their exposure to local IL-4. These macrophages exhibit upregulation of IGF-1, which activates IGF-1R and PI3K signaling in tumor cells and develop tolerance to TAM depletion (90). Besides, a compensatory increased infiltration and activity in MDSCs, cancer associated fibroblasts or neutrophils will contribute to the refractoriness of TAM depleting regimen. Finally, for the bright prospect of TAM intervention, studies are warranted to investigate the tolerability and therapeutic efficacy of TAM-targeted agents in combination with standard and frontline therapies in the clinical arena.
Acknowledgments
Funding: None.
Footnote
Conflicts of Interest: Both authors have completed the ICMJE uniform disclosure form (available at http://dx.doi.org/10.21037/aob.2018.04.04). The authors have no conflicts of interest to declare.
Ethical Statement: The authors are accountable for all aspects of the manuscript and ensure that questions related to the accuracy or integrity of any part of the work are appropriately investigated and resolved.
Open Access Statement: This is an Open Access article distributed in accordance with the Creative Commons Attribution-NonCommercial-NoDerivs 4.0 International License (CC BY-NC-ND 4.0), which permits the non-commercial replication and distribution of the article with the strict proviso that no changes or edits are made and the original work is properly cited (including links to both the formal publication through the relevant DOI and the license). See: https://creativecommons.org/licenses/by-nc-nd/4.0/.
References
- Mantovani A, Allavena P, Sica A, et al. Cancer-related inflammation. Nature 2008;454:436-44. [Crossref] [PubMed]
- Hanahan D, Weinberg RA. Hallmarks of cancer: The next generation. Cell 2011;144:646-74. [Crossref] [PubMed]
- Mantovani A, Marchesi F, Malesci A, et al. Tumour-associated macrophages as treatment targets in oncology. Nat Rev Clin Oncol 2017;14:399-416. [Crossref] [PubMed]
- Wynn TA, Chawla A, Pollard JW. Macrophage biology in development, homeostasis and disease. Nature 2013;496:445-55. [Crossref] [PubMed]
- Franklin RA, Liao W, Sarkar A, et al. The cellular and molecular origin of tumor-associated macrophages. Science 2014;344:921-5. [Crossref] [PubMed]
- Noy R, Pollard JW. Tumor-Associated Macrophages: From Mechanisms to Therapy. Immunity 2014;41:49-61. [Crossref] [PubMed]
- Shibue T, Weinberg RA. EMT, CSCs, and drug resistance: the mechanistic link and clinical implications. Nat Rev Clin Oncol 2017;14:611-29. [Crossref] [PubMed]
- Mani SA, Guo W, Liao MJ, et al. The epithelial-mesenchymal transition generates cells with properties of stem cells. Cell 2008;133:704-15. [Crossref] [PubMed]
- Su S, Liu Q, Chen J, et al. A positive feedback loop between mesenchymal-like cancer cells and macrophages is essential to breast cancer metastasis. Cancer Cell 2014;25:605-20. [Crossref] [PubMed]
- Li HY, Cui XY, Wu W, et al. Pyk2 and Src mediate signaling to CCL18-induced breast cancer metastasis. J Cell Biochem 2014;115:596-603. [Crossref] [PubMed]
- Su S, Wu W, He C, et al. Breaking the vicious cycle between breast cancer cells and tumor-associated macrophages. Oncoimmunology 2014;3:e953418 [Crossref] [PubMed]
- Lin X, Chen L, Yao Y, et al. CCL18-mediated down-regulation of miR98 and miR27b promotes breast cancer metastasis. Oncotarget 2015;6:20485-99. [PubMed]
- Zhang B, Yin C, Li H, et al. Nir1 promotes invasion of breast cancer cells by binding to chemokine (C-C motif) ligand 18 through the PI3K/Akt/GSK3beta/Snail signalling pathway. Eur J Cancer 2013;49:3900-13. [Crossref] [PubMed]
- Scheel C, Eaton Elinor N, Li Sophia HJ, et al. Paracrine and Autocrine Signals Induce and Maintain Mesenchymal and Stem Cell States in the Breast. Cell 2011;145:926-40. [Crossref] [PubMed]
- Chaudhury A, Cheema S, Fachini JM, et al. CELF1 is a central node in post-transcriptional regulatory programmes underlying EMT. Nat Commun 2016;7:13362. [Crossref] [PubMed]
- Romagnoli M, Belguise K, Yu Z, et al. Epithelial-to-Mesenchymal Transition Induced by TGF- 1 Is Mediated by Blimp-1-Dependent Repression of BMP-5. Cancer Res 2012;72:6268-78. [Crossref] [PubMed]
- Zhang J. TGF-β-induced epithelial-to-mesenchymal transition proceeds through stepwise activation of multiple feedback loops. Sci Signal 2014;7:ra91. [Crossref] [PubMed]
- Ni T, Li XY, Lu N, et al. Snail1-dependent p53 repression regulates expansion and activity of tumour-initiating cells in breast cancer. Nat Cell Biol 2016;18:1221-32. [Crossref] [PubMed]
- Akhurst RJ, Hata A. Targeting the TGFbeta signalling pathway in disease. Nat Rev Drug Discov 2012;11:790-811. [Crossref] [PubMed]
- Neuzillet C, Tijeras-Raballand A, Cohen R, et al. Targeting the TGFbeta pathway for cancer therapy. Pharmacol Ther 2015;147:22-31. [Crossref] [PubMed]
- Massagué J. TGFbeta signalling in context. Nat Rev Mol Cell Biol 2012;13:616-30. [Crossref] [PubMed]
- Deheuninck J, Luo K. Ski and SnoN, potent negative regulators of TGF-beta signaling. Cell Res 2009;19:47-57. [Crossref] [PubMed]
- Lu H, Clauser KR, Tam WL, et al. A breast cancer stem cell niche supported by juxtacrine signalling from monocytes and macrophages. Nat Cell Biol 2014;16:1105-17. [Crossref] [PubMed]
- Sangaletti S, Tripodo C, Santangelo A, et al. Mesenchymal Transition of High-Grade Breast Carcinomas Depends on Extracellular Matrix Control of Myeloid Suppressor Cell Activity. Cell Rep 2016;17:233-48. [Crossref] [PubMed]
- Ouzounova M, Lee E, Piranlioglu R, et al. Monocytic and granulocytic myeloid derived suppressor cells differentially regulate spatiotemporal tumour plasticity during metastatic cascade. Nat Commun 2017;8:14979. [Crossref] [PubMed]
- Keskin D, Kim J, Cooke VG, et al. Targeting vascular pericytes in hypoxic tumors increases lung metastasis via angiopoietin-2. Cell Rep 2015;10:1066-81. [Crossref] [PubMed]
- Herbst RS, Hong D, Chap L, et al. Safety, Pharmacokinetics, and Antitumor Activity of AMG 386, a Selective Angiopoietin Inhibitor, in Adult Patients With Advanced Solid Tumors. J Clin Oncol 2009;27:3557-65. [Crossref] [PubMed]
- Bossi P, Viale G, Lee AKC, et al. Angiogenesis in colorectal tumors - microvessel quantitation in adenomas and carcinomas with clinicopathological correlations. Cancer Res 1995;55:5049-53. [PubMed]
- Bergers G, Benjamin LE. Tumorigenesis and the angiogenic switch. Nat Rev Cancer 2003;3:401-10. [Crossref] [PubMed]
- De Palma M, Biziato D, Petrova TV. Microenvironmental regulation of tumour angiogenesis. Nat Rev Cancer 2017;17:457-74. [Crossref] [PubMed]
- Priceman SJ, Sung JL, Shaposhnik Z, et al. Targeting distinct tumor-infiltrating myeloid cells by inhibiting CSF-1 receptor: combating tumor evasion of antiangiogenic therapy. Blood 2010;115:1461-71. [Crossref] [PubMed]
- Potente M, Gerhardt H, Carmeliet P. Basic and Therapeutic Aspects of Angiogenesis. Cell 2011;146:873-87. [Crossref] [PubMed]
- Murdoch C, Muthana M, Coffelt SB, et al. The role of myeloid cells in the promotion of tumour angiogenesis. Nat Rev Cancer 2008;8:618-31. [Crossref] [PubMed]
- Olson OC, Joyce JA. Cysteine cathepsin proteases: regulators of cancer progression and therapeutic response. Nat Rev Cancer 2015;15:712-29. [Crossref] [PubMed]
- Joyce JA, Baruch A, Chehade K, et al. Cathepsin cysteine proteases are effectors of invasive growth and angiogenesis during multistage tumorigenesis. Cancer Cell 2004;5:443-53. [Crossref] [PubMed]
- Giraudo E, Inoue M, Hanahan D. An amino-bisphosphonate targets MMP-9-expressing macrophages and angiogenesis to impair cervical carcinogenesis. J Clin Invest 2004;114:623-33. [Crossref] [PubMed]
- Huang S, Van Arsdall M, Tedjarati S, et al. Contributions of stromal metalloproteinase-9 to angiogenesis and growth of human ovarian carcinoma in mice. J Natl Cancer Inst 2002;94:1134-42. [Crossref] [PubMed]
- De Palma M, Venneri MA, Galli R, et al. Tie2 identifies a hematopoietic monocytes required for tumor lineage of proangiogenic vessel formation and a mesenchymal population of pericyte progenitors. Cancer Cell 2005;8:211-26. [Crossref] [PubMed]
- Chen L, Li J, Wang F, et al. Tie2 Expression on Macrophages Is Required for Blood Vessel Reconstruction and Tumor Relapse after Chemotherapy. Cancer Res 2016;76:6828-38. [Crossref] [PubMed]
- Mazzieri R, Pucci F, Moi D, et al. Targeting the ANG2/TIE2 Axis Inhibits Tumor Growth and Metastasis by Impairing Angiogenesis and Disabling Rebounds of Proangiogenic Myeloid Cells. Cancer Cell 2011;19:512-26. [Crossref] [PubMed]
- Lohela M, Casbon AJ, Olow A, et al. Intravital imaging reveals distinct responses of depleting dynamic tumor-associated macrophage and dendritic cell subpopulations. Proc Natl Acad Sci U S A 2014;111:E5086-95. [Crossref] [PubMed]
- Clear AJ, Lee AM, Calaminici M, et al. Increased angiogenic sprouting in poor prognosis FL is associated with elevated numbers of CD163(+) macrophages within the immediate sprouting microenvironment. Blood 2010;115:5053-6. [Crossref] [PubMed]
- Stockmann C, Doedens A, Weidemann A, et al. Deletion of vascular endothelial growth factor in myeloid cells accelerates tumorigenesis. Nature 2008;456:814-8. [Crossref] [PubMed]
- Shojaei F, Wu X, Zhong C, et al. Bv8 regulates myeloid-cell-dependent tumour angiogenesis. Nature 2007;450:825-31. [Crossref] [PubMed]
- Tian L, Goldstein A, Wang H, et al. Mutual regulation of tumour vessel normalization and immunostimulatory reprogramming. Nature 2017;544:250-4. [Crossref] [PubMed]
- Schmittnaegel M, Rigamonti N, Kadioglu E, et al. Dual angiopoietin-2 and VEGFA inhibition elicits antitumor immunity that is enhanced by PD-1 checkpoint blockade. Sci Transl Med 2017;9: [Crossref] [PubMed]
- Peinado H, Zhang H, Matei IR, et al. Pre-metastatic niches: organ-specific homes for metastases. Nat Rev Cancer 2017;17:302-17. [Crossref] [PubMed]
- Liu Y, Cao X. Characteristics and Significance of the Pre-metastatic Niche. Cancer Cell 2016;30:668-81. [Crossref] [PubMed]
- Sleeman JP. The lymph node pre-metastatic niche. J Mol Med (Berl) 2015;93:1173-84. [Crossref] [PubMed]
- Hiratsuka S, Ishibashi S, Tomita T, et al. Primary tumours modulate innate immune signalling to create pre-metastatic vascular hyperpermeability foci. Nat Commun 2013;4:1853. [Crossref] [PubMed]
- Hiratsuka S, Watanabe A, Sakurai Y, et al. The S100A8-serum amyloid A3-TLR4 paracrine cascade establishes a pre-metastatic phase. Nat Cell Biol 2008;10:1349-55. [Crossref] [PubMed]
- Yan HH, Pickup M, Pang Y, et al. Gr-1+CD11b+ myeloid cells tip the balance of immune protection to tumor promotion in the premetastatic lung. Cancer Res 2010;70:6139-49. [Crossref] [PubMed]
- Costa-Silva B, Aiello NM, Ocean AJ, et al. Pancreatic cancer exosomes initiate pre-metastatic niche formation in the liver. Nat Cell Biol 2015;17:816-26. [Crossref] [PubMed]
- Erler JT, Bennewith KL, Cox TR, et al. Hypoxia-Induced Lysyl Oxidase Is a Critical Mediator of Bone Marrow Cell Recruitment to Form the Premetastatic Niche. Cancer Cell 2009;15:35-44. [Crossref] [PubMed]
- Cox TR, Rumney RM, Schoof EM, et al. The hypoxic cancer secretome induces pre-metastatic bone lesions through lysyl oxidase. Nature 2015;522:106-10. [Crossref] [PubMed]
- Su S, Zhao Q, He C, et al. miR-142-5p and miR-130a-3p are regulated by IL-4 and IL-13 and control profibrogenic macrophage program. Nat Commun 2015;6:8523. [Crossref] [PubMed]
- Liu Z, Hou J, Ren L, et al. Protein tyrosine phosphatase receptor type O expression in the tumor niche correlates with reduced tumor growth, angiogenesis, circulating tumor cells and metastasis of breast cancer. Oncol Rep 2015;33:1908-14. [Crossref] [PubMed]
- Headley MB, Bins A, Nip A, et al. Visualization of immediate immune responses to pioneer metastatic cells in the lung. Nature 2016;531:513-7. [Crossref] [PubMed]
- Song KH, Park MS, Nandu TS, et al. GALNT14 promotes lung-specific breast cancer metastasis by modulating self-renewal and interaction with the lung microenvironment. Nat Commun 2016;7:13796. [Crossref] [PubMed]
- Acharyya S, Oskarsson T, Vanharanta S, et al. A CXCL1 paracrine network links cancer chemoresistance and metastasis. Cell 2012;150:165-78. [Crossref] [PubMed]
- Qian BZ, Zhang H, Li J, et al. FLT1 signaling in metastasis-associated macrophages activates an inflammatory signature that promotes breast cancer metastasis. J Exp Med 2015;212:1433-48. [Crossref] [PubMed]
- Mathsyaraja H, Thies K, Taffany DA, et al. CSF1-ETS2-induced microRNA in myeloid cells promote metastatic tumor growth. Oncogene 2015;34:3651-61. [Crossref] [PubMed]
- Lobera M, Madauss KP, Pohlhaus DT, et al. Selective class IIa histone deacetylase inhibition via a nonchelating zinc-binding group. Nat Chem Biol 2013;9:319-25. [Crossref] [PubMed]
- Guerriero JL, Sotayo A, Ponichtera HE, et al. Class IIa HDAC inhibition reduces breast tumours and metastases through anti-tumour macrophages. Nature 2017;543:428-32. [Crossref] [PubMed]
- Stankic M, Pavlovic S, Chin Y, et al. TGF-beta-Id1 signaling opposes Twist1 and promotes metastatic colonization via a mesenchymal-to-epithelial transition. Cell Rep 2013;5:1228-42. [Crossref] [PubMed]
- Gao D, Joshi N, Choi H, et al. Myeloid progenitor cells in the premetastatic lung promote metastases by inducing mesenchymal to epithelial transition. Cancer Res 2012;72:1384-94. [Crossref] [PubMed]
- Tang H, Leung L, Saturno G, et al. Lysyl oxidase drives tumour progression by trapping EGF receptors at the cell surface. Nat Commun 2017;8:14909. [Crossref] [PubMed]
- Oskarsson T, Acharyya S, Zhang XH, et al. Breast cancer cells produce tenascin C as a metastatic niche component to colonize the lungs. Nat Med 2011;17:867-74. [Crossref] [PubMed]
- Zhang XH, Wang Q, Gerald W, et al. Latent bone metastasis in breast cancer tied to Src-dependent survival signals. Cancer Cell 2009;16:67-78. [Crossref] [PubMed]
- Lu X, Mu E, Wei Y, et al. VCAM-1 Promotes Osteolytic Expansion of Indolent Bone Micrometastasis of Breast Cancer by Engaging α4β1-Positive Osteoclast Progenitors. Cancer Cell 2011;20:701-14. [Crossref] [PubMed]
- Hughes R, Qian BZ, Rowan C, et al. Perivascular M2 macrophages stimulate tumor relapse after chemotherapy. Cancer Res 2015;75:3479-91. [Crossref] [PubMed]
- Kozin SV, Kamoun WS, Huang Y, et al. Recruitment of myeloid but not endothelial precursor cells facilitates tumor regrowth after local irradiation. Cancer Res 2010;70:5679-85. [Crossref] [PubMed]
- Kitamura T, Qian BZ, Soong D, et al. CCL2-induced chemokine cascade promotes breast cancer metastasis by enhancing retention of metastasis-associated macrophages. J Exp Med 2015;212:1043-59. [Crossref] [PubMed]
- De Palma M, Lewis CE. Macrophage regulation of tumor responses to anticancer therapies. Cancer Cell 2013;23:277-86. [Crossref] [PubMed]
- Yap TA, Smith AD, Ferraldeschi R, et al. Drug discovery in advanced prostate cancer: translating biology into therapy. Nat Rev Drug Discov 2016;15:699-718. [Crossref] [PubMed]
- Ma CX, Reinert T, Chmielewska I, et al. Mechanisms of aromatase inhibitor resistance. Nat Rev Cancer 2015;15:261-75. [Crossref] [PubMed]
- Lu X, Horner JW, Paul E, et al. Effective combinatorial immunotherapy for castration-resistant prostate cancer. Nature 2017;543:728-32. [Crossref] [PubMed]
- Stender JD, Nwachukwu JC, Kastrati I, et al. Structural and Molecular Mechanisms of Cytokine-Mediated Endocrine Resistance in Human Breast Cancer Cells. Mol Cell 2017;65:1122-1135.e5. [Crossref] [PubMed]
- Franco HL, Nagari A, Kraus WL. TNFalpha signaling exposes latent estrogen receptor binding sites to alter the breast cancer cell transcriptome. Mol Cell 2015;58:21-34. [Crossref] [PubMed]
- Hiken JF, McDonald JI, Decker KF, et al. Epigenetic activation of the prostaglandin receptor EP4 promotes resistance to endocrine therapy for breast cancer. Oncogene 2017;36:2319-27. [Crossref] [PubMed]
- Fan P, Wang J, Santen RJ, et al. Long-term Treatment with Tamoxifen Facilitates Translocation of Estrogen Receptor out of the Nucleus and Enhances its Interaction with EGFR in MCF-7 Breast Cancer Cells. Cancer Res 2007;67:1352-60. [Crossref] [PubMed]
- Turner N, Pearson A, Sharpe R, et al. FGFR1 amplification drives endocrine therapy resistance and is a therapeutic target in breast cancer. Cancer Res 2010;70:2085-94. [Crossref] [PubMed]
- Stossi F, Madak-Erdogan Z, Katzenellenbogen BS. Macrophage-elicited loss of estrogen receptor-alpha in breast cancer cells via involvement of MAPK and c-Jun at the ESR1 genomic locus. Oncogene 2012;31:1825-34. [Crossref] [PubMed]
- Miller TW, Hennessy BT, Gonzalez-Angulo AM, et al. Hyperactivation of phosphatidylinositol-3 kinase promotes escape from hormone dependence in estrogen receptor-positive human breast cancer. J Clin Invest 2010;120:2406-13. [Crossref] [PubMed]
- Carver BS, Chapinski C, Wongvipat J, et al. Reciprocal feedback regulation of PI3K and androgen receptor signaling in PTEN-deficient prostate cancer. Cancer Cell 2011;19:575-86. [Crossref] [PubMed]
- Schwartz S, Wongvipat J, Trigwell CB, et al. Feedback suppression of PI3Kalpha signaling in PTEN-mutated tumors is relieved by selective inhibition of PI3Kbeta. Cancer Cell 2015;27:109-22. [Crossref] [PubMed]
- Tap WD, Wainberg ZA, Anthony SP, et al. Structure-Guided Blockade of CSF1R Kinase in Tenosynovial Giant-Cell Tumor. N Engl J Med 2015;373:428-37. [Crossref] [PubMed]
- Nywening TM, Wang-Gillam A, Sanford DE, et al. Targeting tumour-associated macrophages with CCR2 inhibition in combination with FOLFIRINOX in patients with borderline resectable and locally advanced pancreatic cancer: a single-centre, open-label, dose-finding, non-randomised, phase 1b trial. Lancet Oncol 2016;17:651-62. [Crossref] [PubMed]
- Butowski N, Colman H, De Groot JF, et al. Orally administered colony stimulating factor 1 receptor inhibitor PLX3397 in recurrent glioblastoma: an Ivy Foundation Early Phase Clinical Trials Consortium phase II study. Neuro Oncol 2016;18:557-64. [Crossref] [PubMed]
- Quail DF, Bowman RL, Akkari L, et al. The tumor microenvironment underlies acquired resistance to CSF-1R inhibition in gliomas. Science 2016;352:aad3018 [Crossref] [PubMed]
Cite this article as: Lao L, Song E. Therapeutic potential of tumor associated macrophages. Ann Blood 2018;3:27.