A review of the pathophysiology of paroxysmal nocturnal hemoglobinuria
Introduction
Paroxysmal nocturnal hemoglobinuria (PNH) is a complex disease which runs a devastating course and carries a median untreated survival from diagnosis of 10 years (1). It has a multifaceted pathophysiology which incorporates genetic mutations and polymorphisms, the complement cascade and relentless intravascular hemolysis. There is a significant overlap with bone marrow failure and there remains a risk of clonal evolution. The understanding of PNH has improved dramatically over recent decades and this review aims to provide a comprehensive overview of the latest insights into its pathophysiology, focusing on the normal function and regulation of the complement system, the cellular and molecular mechanisms underlying PNH, as well as the clinical manifestations of the disease. Additionally, the review will discuss the therapeutic landscape of PNH including the mechanisms of available complement inhibitors and factors influencing patient-to-patient variability in treatment responses, the latter of which has not been covered in other reviews.
Normal complement function
The complement system (or cascade) is an integral part of the innate immune system, evolved primarily to protect us against invading pathogens. It is composed of over 50 proteins (2) which interact, cleave and combine to complement antibodies in our defences, particularly against encapsulated bacteria such as Hemophilus influenzae, Neisseria meningitidis and Streptococcus pneumoniae (3). It has three canonical pathways of activation, classical (CP), lectin (LP) and alternative (AP), which converge at the enzymatic activation of complement component C3. The nomenclature of complement proteins, which numerically begin at C1 and end at C9, inconveniently reflect their chronological order of discovery rather than the order in which they appear in the cascade (3) (Figure 1).
The CP was the first pathway to be discovered and is typically initiated when antibody complexes bind to invading pathogens, activating complement component C1q (3). C1q binds to the Fc portion of a single surface-bound IgM, or at least two IgG antibodies in close proximity, and undergoes a conformational change. This change allows C1q to activate C1r and C1s, leading to cleavage of complement component C4 into C4a and C4b. The lectin pathway is similar except serum proteins mannose-binding lectin (MBL) and ficolins bind directly to the bacterial surface by attaching to carbohydrates among other binding partners (4). This is recognised by MBL-associated proteases (MASP-1, MASP-2, MASP-3) which bind to the MBL or ficolin to create a C1-like complex of MBL-MASP (4). This complex behaves like C1 and cleaves C4 into C4a and C4b (4), and from here the two pathways converge. C4b has a thioester which allows it to become covalently bound to the activating surface where it is able to bind to complement component C2 (4). C4b-bound C2 is cleaved by the activated C1 complex into C2a and C2b. The smaller split product C2b (traditional nomenclature denotes the smaller C2 fragment to be C2b) diffuses away, while C2a remains bound to C4b forming the C3 convertase of the CP, C4bC2a. This C3 convertase cleaves C3 into C3a and C3b before binding to the cleavage product C3b to form the C5 convertase of the CP, C4bC2aC3b (3,5,6). This C5 convertase subsequently feeds into the terminal complement pathway.
In the AP, continuous hydrolysis of C3 to C3(H2O) ensures that the AP is always active. Hydrolyzed C3 binds to factor B (FB) which is in turn cleaved by factor D (FD) [itself formed when pro-FD is activated by MASP-3 (7)], with the resultant product being a C3 ‘initiation convertase’, C3(H2O)Bb. This C3 convertase cleaves C3 to C3a (an anaphylatoxin) and C3b, the latter of which (like C4b) has a thioester which can bind covalently to the activating surface. C3b also has a binding site for FB, and when C3b-bound FB is cleaved by FD, the resultant molecule C3bBb is the C3 convertase of the AP. C3bBb is stabilized by the binding of the protein properdin (3). C3bBb cleaves C3 into C3b which itself forms new C3bBb molecules; an ‘amplification loop’ which allows for a rapid upregulation of complement in response to infection. A further single C3b molecule can bind to C3bBb to form the C5 convertase of the AP, C3bBbC3b (3).
As well as being vital for the production of C3 and C5 convertases, the opsonization of pathogens with covalently bound C3b and C4b also promotes phagocytosis in the spleen (4).
All three of the above pathways result in the formation of a C5 convertase on the surface of the invading bacteria, marking the start of the terminal pathway where C5 is cleaved into C5a (a powerful anaphylatoxin) and C5b. The C5b molecule recruits C6 forming C5b,6 which in turn recruits C6, C7, C8 and multiple C9 molecules to form a membrane attack complex (MAC), a transmembrane pore which causes osmotic lysis of the targeted cell (3).
Although this has long been the accepted tenet of the complement cascade, recent studies suggest an alternative mechanism at the level of the terminal pathway. The concept of a trimolecular C5 convertase has been disputed, as C3b is unable to bind in vitro to immobilized C3bBb (8), a process required to form the C5 convertase C3bBbC3b. Instead, it is suggested that as C3b accumulates on the cell surface it recruits and ‘primes’ C5 molecules for cleavage by the C3 convertases (8-12).
Normal complement regulation
An important aspect of the complement system is to protect healthy host cells against complement-mediated damage, and to downregulate when no longer required. There are dozens of regulators in action which work together in a 3-stage strategy: (I) limit activation of complement when not required (or moderate activation when no strong activation is needed), (II) regulate complement convertases (which are central amplifiers) on host surfaces, and (III) prevent MAC formation on host surfaces (13).
C1 inhibitor (C1-INH) is the main regulator controlling activation of the CP and LP. It is an inhibitor which irreversibly binds to the catalytic centre of C1r and C1s, preventing them from cleaving C4 (14), blocking the CP before the formation of the C3 convertase. C1-INH also inhibits MASP proteases and thus downregulates the LP, although there are other proteins (sMAP, MAP-1) which limit the LP by competing for binding sites on MBL and ficolins (14).
The main regulator of C3b, C4b, and the convertases is Factor I (FI) (Figure 2). This circulating protein cooperates with so-called cofactor proteins to cleave C3b and C4b into inactive products which can no longer form convertases (iC3b/C3dg, iC4b/C4d) (14). FI works alongside a family of six regulatory proteins called ‘regulators of complement activity’ (RCA). These proteins are all encoded on chromosome 1q32 (15) and consist of three membrane-bound components [complement receptor 1 (CR1; or CD35), membrane cofactor protein (MCP; or CD46) and decay-accelerating factor (CD55)] and three soluble components [C4b-binding protein (C4BP), Factor H (FH) and its alternative splice product Factor H-like 1 (FHL-1)] (14). The RCA proteins have either cofactor activity or decay accelerating activity or both. Cofactors assist Factor I in proteolytically trimming C3b and C4b. Decay accelerators prevent the assembly of convertases or decay them if already formed.
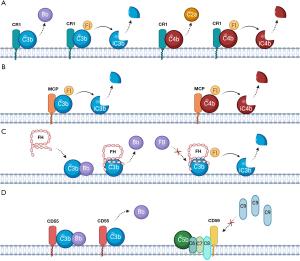
CR1 (CD35) is a glycoprotein expressed on most blood cells which has a number of binding sites for C3b and C4b, acting as a co-factor to increase their FI-mediated inactivation (Figure 2). It is involved in the decay of C3 and C5 convertases (5) and transporting C3b and/or C4b-loaded particles to the spleen for phagocytosis, a phenomenon which is called immune adherence (14). MCP (or CD46), widely expressed on most cell surfaces except red blood cells (RBCs), is another cofactor to FI (14). CD55 is expressed on almost all peripheral blood cells and C5 convertases, and accelerates their decay (4) (Figure 2). While CR1, CD55 and MCP regulate the CP/LP and AP, the two remaining RCA proteins are specific for different activation paths. C4BP is CP specific; it is another cofactor for FI and accelerates the natural decay of C3-convertase (16).
FH is the main fluid phase regulator of the AP. It binds to C3b, competing with complement FB, blocking C3bBb (C3 convertase) formation. Furthermore, it accelerates the irreversible decay of C3 convertases that do form, and acts as a co-factor for factor I (17) (Figure 2). FHL-1 is an alternative splice-product which shares the same gene as FH, produced as a truncated form of the protein with similar function (6).
Finally in the terminal pathway, CD59 is an important cell surface regulator which binds to C8 or C9 within a C5b-8 or C5b-9 complex and prevents C9 polymerization, inhibiting MAC formation on host cells (14) (Figure 2). Within the serum, clusterin and vitronectin are proteins which form complexes with free C5b-7 to prevent the insertion of developing MAC into bystander cell membranes (5).
PNH at a cellular level
With an understanding of the complement system and its regulation, one can better understand the pathophysiology of PNH. At a cellular level, the key issue is the loss of the complement regulators CD55 and CD59 from cell surfaces. This occurs in hematopoietic stem cells (HSCs) and so all peripheral blood cells derived from those affected HSCs carry this CD55 and CD59 deficiency. Without these protective membrane bound regulators, complement activation and amplification is under-regulated on host cells. On affected RBCs, this means that already weak to moderate complement activation triggers may lead to MAC formation and chronic intravascular hemolysis. RBCs are particularly vulnerable as the loss of CD55 and CD59 is compounded by the natural absence of the complement regulator MCP (14).
Outside of PNH, isolated deficiencies of CD55 and CD59 can be seen as inherited disorders. CD55 deficiency with hyperactivation of complement, angiopathic thrombosis and severe protein-losing enteropathy (CHAPLE) syndrome, an inherited CD55 deficiency, doesn’t lead to the development of devastating intravascular hemolysis as the ongoing protection from intact CD59 is sufficient (18). In contrast, patients with an inherited CD59 deficiency present with chronic and paroxysmal intravascular hemolysis akin to PNH, though it is seen in association with a chronic inflammatory demyelinating polyradiculoneuropathy (19).
CD55 and CD59 deficiency in PNH stems from an error in the surface expression of the anchor required to bind these proteins to the cell membrane. The anchor in question is glycophosphatidylinositol (GPI), and over 150 GPI anchored proteins (GPI-AP) have been identified (20).
In PNH, the amount of CD55 and CD59 seen on RBC surfaces can vary. Cells which have a complete deficiency are labelled Type III PNH cells, whilst those which only have a partial deficiency are Type II PNH cells (21). Type I PNH cells are normal RBCs with normal CD55 and CD59 expression. Type III cells are 10–15× more sensitive to complement-mediated hemolysis than Type I cells, whilst Type II cells are 3–5× more sensitive (21). Generally, the larger the proportion of Type III cells the more severe the hemolysis a patient experiences.
As well as the absence of GPI-AP, PNH at a cellular level may involve the metabolomic profile; the small molecules produced during cellular metabolism (22). Similar to other hemolytic anemias, patients with PNH have been found to have an altered metabolome compared with healthy controls, with lower levels of amino acids participating in the glycogenesis pathway with impaired glutaminolysis (22). The degree (if any) to which the metabolome is involved in the pathogenesis of PNH, the expansion of a PNH clone or individual responses to complement inhibitors is yet to be determined and is an area of ongoing research (23,24).
PNH at a molecular level
GPI-AP biosynthesis is encoded by at least 33 genes and a 23-step engineering process (25) which involves combining sugar nucleotides and phospholipids within the endoplasmic reticulum, before transfer of the completed molecule to the cell surface (26). One of these 33 genes, phosphatidylinositol N-acetylglucosaminyltransferase subunit A (PIGA), is responsible for producing a 484 amino-acid protein required in the early stages of GPI-AP synthesis (27,28). PNH is classically caused by acquired somatic mutations in the PIGA gene (29).
The PIGA gene is located on the X chromosome, though mutations in PNH develop after X-chromosome inactivation, explaining why PNH is seen in equal number of males and females (28) and why a mutation in only a single gene is required for the PNH phenotype to appear (28). Hundreds of different mutations in the PIGA gene have been identified and these can be insertions, deletions, missense or nonsense (28). Missense mutations may leave some residual PIGA activity, explaining the presence of Type II PNH cells (28). Patients can have multiple populations of PNH clones, each with different PIGA mutations (30).
Despite its recognized association, the relationship between PIGA mutations and PNH is complex. Firstly, although the large majority of patients with PNH have a PIGA mutation, it can be absent in some. For these patients, it may mean mutations in other genes encoding for GPI-AP biosynthesis, and pathogenic mutations of PIGT and PIGV have been reported in patients with normal PIGA genes (31). What is more, the presence of a PIGA gene mutation cannot be said to be pathognomonic for PNH, with mutations identifiable in the healthy population. These are associated with very small asymptomatic PNH clones of <0.001% and are detectable in polyclonal colony-forming cells which, unlike HSCs, do not have the capacity to self-renew (32,33).
PNH clone expansion and bone marrow failure (BMF)
What continues to elude researchers is how PNH cells proliferate in preference to normal HSCs, and what drives the PNH clone to expand in some patients but not in others. PNH cells have no intrinsic growth advantage over normal HSCs (34), which explains how PIGA mutations can be detected in healthy donors with no sequalae of PNH. PNH is strongly associated with aplastic anemia (AA) and it is widely accepted that PNH develops on a background of BMF (35). In this context it is acknowledged that PNH cells likely escape autoreactive CD8+ T-lymphocytes (which cause AA), through the loss of GPI-AP bound co-stimulatory molecules or autoantigens in the bone marrow (36); an escape model in which the PNH clone is not susceptible to autoimmune attack, potentially by losing the direct target of the T-cells, GPI itself (37). As for PNH clone expansion, it may in part be related to other genetic mutations within the HSCs; mouse models have shown that increased HMGA2 expression is associated with clonal expansion and this can be seen in higher levels in patients with PNH (38). It has previously been suggested that PNH cells are more resistant to apoptosis than GPI-AP positive cells, proposing a mechanism of clonal expansion, although in vitro data are contradictory (39).
Clinical features of PNH
The most common clinical features at the time of PNH diagnosis are anemia, fatigue, hemoglobinuria, breathlessness, thrombosis, abdominal pain, dysphagia and, in men, erectile dysfunction (40).
Anemia in PNH is likely multifactorial, with an increase in RBC destruction through chronic intravascular hemolysis being compounded by impaired RBC production due to urinary iron loss (41) and BMF. Breathlessness can in part be attributed to anemia but patients are also at risk of pulmonary hypertension. Free hemoglobin in the serum, released during intravascular hemolysis, reacts with and destroys nitric oxide (NO), a potent endothelium-derived relaxing factor. NO relaxes smooth muscle and induces vasodilation, with chronic NO scavenging by hemoglobin causing marked vasoconstriction (42). Vasoconstriction within the lungs leads to pulmonary hypertension, whilst in the kidneys it can contribute to renal disease. Renal impairment can be compounded by damage to the renal tubules through chronic iron deposition, the toxicity of free hemoglobin and repeated microvascular thrombosis (43). Renal vein or artery thrombosis is rare (43). NO depletion and impairment of smooth muscle relaxation further leads to abdominal pain, dysphagia and erectile dysfunction.
Thrombosis in PNH is complex, resulting from the overlap of the complement and clotting cascades (Figure 3) (44). Without the protection of CD59, PNH platelets are susceptible to constant complement-mediated activation, with clotting further stimulated by free hemoglobin and NO depletion. Activated circulating PNH platelets release prothrombotic microvesicles which act as binding sites for prothrombinase and tenase, upregulating the clotting cascade and stimulating thrombin production (44). Thrombin cleaves fibrinogen to form a fibrin clot which is stabilized by the activity of Factor XIII. Factor XIII is inhibited by NO, so NO deficiency in PNH may lead to increased clot stability (45). Furthermore, PNH white blood cells lose the GPI-AP, urokinase-type plasminogen activator receptor (u-PAR) and tissue factor pathway inhibitor (TFPI). These are natural anticoagulants which increase clot lysis (uPAR) and inhibit factor X and tissue factor-VIIa complexes (TFPI), and their absence may promote clot formation (44). Adding to this hypercoagulable state, the proinflammatory anaphylatoxin C5a promotes vasoconstriction in an already NO deplete vasculature, leading to impaired hemodynamic flow (44). Completing Virchow’s triad, free heme released during hemolysis is also thought to irritate the vascular endothelium, leading to local injury and inflammation (46). One of the most common sites of thrombosis for patients with PNH is the hepatic vein (Budd-Chiari syndrome), although the mechanism behind the predilection for this site is not known (40). The use of anticoagulation alone in patients with PNH is not always enough to prevent Budd-Chiari syndrome (47).
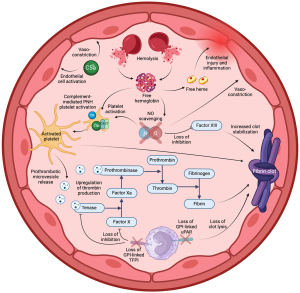
Unsurprisingly, patients at the highest risk of thrombosis are those with previous thromboses, high white blood cell PNH clones and evidence of high disease activity (48), but there are cohorts of patients with atypical PNH clones who also appear to be at high risk. Patients with a high white blood cell clone but low red cell clone, and those with a higher Type II clone than Type III clone both appear to be at an increased risk and this reflects how non-hemolytic mechanisms play a major role in thrombosis (49).
Current treatments
Until the early 2000s, treatment for PNH was supportive; blood transfusions, iron and folate replacement, and anticoagulation. For many patients globally who do not have access to complement inhibitors, this unfortunately remains the mainstay of treatment. 2002 saw the first pilot study of eculizumab in the treatment of PNH and its success led to eculizumab being the gold standard therapy (50).
Eculizumab is a humanized monoclonal IgG antibody which specifically binds with high-affinity to C5, blocking its cleavage (50). In doing so, it prevents the generation of C5b required for MAC formation. Eculizumab specifically binds to the macroglobulin MG7 domain of C5, though a rare subset of Japanese patients with a polymorphism in Arg885 have an altered epitope to which eculizumab is unable to bind, leading to treatment failure (51). Eculizumab is administered intravenously every 2 weeks. Biosimilars to eculizumab are now available which have the same mechanism of action and offer non-inferiority (52).
Ravulizumab is a humanized monoclonal IgG antibody directed against C5, bioengineered from eculizumab to increase its half-life fourfold, allowing it to be administered intravenously every 8 weeks. By altering the amino acid sequence in the complementary determining and neonatal Fc-receptor-binding regions of the eculizumab molecule, there is increased dissociation of C5 from ravulizumab in the acidic environment of the intracellular endosome as well as improved recycling via the neonatal Fc-receptor. As such, the amount of antibody that undergoes lysosomal degradation in vascular endothelial cells is reduced, increasing the amount of free antibody released back into the circulation which can then rebind to C5, allowing for less frequent dosing (53,54).
The introduction of both eculizumab and ravulizumab into the treatment plans of patients with PNH had a revolutionary impact on their morbidity and mortality, with those patients now having the same life-expectancy as age- and sex-matched controls (excluding those who require treatment for BMF) (40).
Other available C5 inhibitors include crovalimab and pozelimab. Crovalimab is a humanized monoclonal IgG which, like ravulizumab, is recycled efficiently from the acidic endosome and returned into the circulation (55), though it targets a different C5 epitope to that of eculizumab and ravulizumab (56). Crovalimab is given as a subcutaneous injection every 4 weeks (56). Pozelimab is a monoclonal antibody given as a weekly subcutaneous infusion, after initial intravenous loading (57). In clinical trials, pozelimab is given alongside cemdisiran, a ’small interfering RNA’ (siRNA) which reduces hepatic C5 production (57).
Moving from terminal inhibition to proximal, pegcetacoplan is a pegylated pentadecapeptide inhibitor of C3, administered as a subcutaneous infusion twice-weekly (58). By blocking the cleavage of C3, pegcetacoplan is an effective inhibitor of the AP, preventing the formation of AP C3 and C5 convertases through the absence of C3b. By blocking C3, pegcetacoplan can be used successfully in patients susceptible to extravascular hemolysis seen as a result of C5 inhibition (59).
Some of the available agents which block proximal to C3 include iptacopan, danicopan and OMS906. Iptacopan (60) and danicopan (61) are both oral small-molecule inhibitors which block FB and FD respectively, both suppressing the AP and the amplification loop. OMS906 is a subcutaneous inhibitor of MASP-3, blocking the AP by reducing pro-FD activation and therefore available FD (62).
A summary of currently available complement inhibitors and their targets within the complement cascade is shown in Table 1.
Table 1
Complement inhibitor | Complement target |
---|---|
Eculizumab (and biosimilars) | C5 |
Ravulizumab | C5 |
Crovalimab | C5 |
Pozelimab | C5 |
Cemdisiran | C5 |
Pegcetacoplan | C3 |
Iptacopan | Factor B |
Danicopan | Factor D |
OMS906 | MASP-3 |
Complement regulators and treatment responses
As described earlier, the RBC antigen CR1 binds to C3b and C4b and acts as a co-factor to increase their FI-mediated inactivation (5). There are two co-dominant alleles that control expression of CR1, labelled H (high expression) and L (low expression) (63). Expressing two alleles, patients can either have the genotype H/H with high levels of CR1, the genotype L/L with low levels of CR1, or have intermediate amounts with the genotypes H/L (63). PNH patients with L/L (and therefore the lowest surface levels of CR1) have the highest levels of C3 deposition, likely due to reduced FI-mediated clearance and lower decay accelerating activity. Clinically, those patients with the L/L polymorphism are seven times more likely to have a suboptimal response to eculizumab (defined as ongoing transfusion requirement) than patients with the H/H phenotype (63).
With FH, gene polymorphisms are more common in patients with PNH than in healthy controls. Patients with atypical variants are more likely to be transfusion dependent at 6 months after starting eculizumab than those who have typical FH genes (64). PNH RBCs have significantly lower surface levels of FH than normal RBCs, thought to be related to lower levels of sialic acids (ligands which help bind FH to the RBC surface) and augmenting surface levels in vitro protects PNH RBCs from complement-mediated lysis (65). FH polymorphisms may therefore play a role in PNH presentation and treatment responses.
Although data on FI polymorphisms in PNH is lacking, a number of polymorphisms have been reported in patients with other complement-mediated disorders such as age-related macular degeneration, C3 glomerulopathy and atypical hemolytic uraemic syndrome (66). More than half of these polymorphisms correlate with reduced levels of FI (66) which one could hypothesise may correlate with higher levels of C3 and C4 deposition, potentially affecting response to complement inhibitors.
Pathophysiology of treatment sequelae
Infection
By blocking the innate immune response, infection has long been a concern for patients receiving a complement inhibitor. The major risk is that of encapsulated bacteria (Streptococcus pneumoniae, Neisseria meningitidis, Hemophilus influenza) which can no longer be destroyed by MAC. Eculizumab carries a 1,000–2,000 fold increased risk of meningococcal infection (67), and patients on C5 inhibitors are required to comply with meningococcal vaccination and, in some countries, regular antibiotic prophylaxis (68). With these measures the rate of meningococcal infection in patients receiving C5 inhibitors is around 0.35 per 100 patient years, although caution is still required; in 509 patients treated with either eculizumab or ravulizumab, there was 1 fatality from 11 cases of meningococcal sepsis over a 20 year period (40). As well as preventing MAC formation, C5 inhibition prevents the production of C5a, a powerful anaphylatoxin which supports the recruitment and activation of granulocytes (4), including the release of proinflammatory mediators (69). Proximal inhibitors will similarly reduce the availability of the anaphylatoxin C3a, though this is less potent than C5a (4). Data on the risk of non-meningococcal infection in patients receiving complement inhibitors versus healthy controls is lacking. One could look to increased infection risk in patients with inherited deficiencies of complement components for comparison (70), though this data is likely to be affected by selection bias. Patient numbers in these reports are low, and it is likely that these patients are discovered to have complement deficiency because of recurrent infections, and we do not know about the possible prevalence of complement deficiencies which may remain asymptomatic. In a Dutch study of patients with PNH receiving eculizumab, the incidence of non-meningococcal infection was 0.91 per year, and the vast majority of these were grade 2 (71); moderate symptomatology limiting important activities of daily living, but not severe enough to limit self-care. How this compares to age- and sex-matched controls is unknown. Confounding the data is the fact that these patients with PNH often have underlying bone marrow failure which contributes to infection risk.
Extravascular hemolysis
A recognised mechanistic consequence of receiving a C5 inhibitor is the surface loading of C3 onto PNH RBCs (72). C3b binds to all PNH RBCs cells due to an absence of CD55, but in untreated patients these cells undergo rapid MAC-mediated hemolysis due to the absence of CD59; when C5 is blocked by C5 inhibitors, these C3-loaded cells avoid MAC-mediated hemolysis. FI together with the required cofactors of the RCA family ensure that C3 is cleaved to C3b (and subsequent degradation products) and these opsonized cells are destroyed through macrophage-mediated extravascular hemolysis in the liver and spleen. Extravascular hemolysis is not as rapid as intravascular hemolysis, allowing C3-loaded cells to be detected in the circulation (72). Approximately 1 in 4 patients receiving a C5 inhibitor have ongoing transfusion requirements (40) and a majority have a hemoglobin below 120 g/L (73), in part due to this extravascular hemolysis, and is a common reason for switching to a proximal complement inhibitor. Patients at highest risk for extravascular hemolysis are probably those with impaired activity of complement regulators.
Breakthrough hemolysis (BTH)
Separate from extravascular hemolysis is BTH which can occur with any complement inhibitor. There has been no consensus on the definition of BTH, but generally includes the development of new or worsening signs or symptoms of intravascular hemolysis in the presence of an elevated lactate dehydrogenase (74). The mechanism of BTH is classed as being either pharmacokinetic or pharmacodynamic.
Pharmacokinetic BTH occurs when the amount of complement inhibitor is subtherapeutic for the levels of available complement, with surplus free complement leading to MAC formation (75). This is commonly seen in complement amplifying conditions such as infections, though could also be caused by missed doses of complement inhibitors. Pharmacodynamic BTH occurs in patients who have seemingly complete blockade of complement, and its mechanism is poorly understood. There is a body of evidence to suggest that pharmacodynamic BTH may occur in the context of PNH RBCs which are densely loaded with either C3b or C4b. In hemolysis assays, RBCs with higher surface densities of C3b demonstrate higher levels of hemolysis, which is not completely blocked by the addition of a C5 inhibitor (11). Furthermore, in vitro studies suggest that when C5 interacts with a RBC surface densely loaded with either C3b or C4b, it undergoes a conformational change in its structure to form a new molecule termed C5conf. This conformational change exposes new binding sites which allow it to form MAC complexes with C6-9 despite not being cleaved to C5b (8). C5conf-mediated MAC formation can be hindered but not completely blocked by the addition of a single C5 inhibitor (8).
Additionally, reactive lysis (Figure 4) is the mechanism by which MAC can be formed on PNH RBCs which haven’t had initial activation of the complement cascade on their surface. When RBCs have undergone MAC-mediated lysis, C5b6 which hasn’t yet generated MAC can be released into the surrounding environment. These C5b6 molecules can bind to ‘bystander’ PNH RBCs and recruit C7, C8 and C9 to create MAC. This has been shown in vitro (76) and the hemolysis is more pronounced in Type III PNH cells than Type II; unsurprising given the protection offered by low levels of CD59. Reactive lysis is also inhibited to a certain degree by complement regulators clusterin and vitronectin (5). Reactive lysis does not explain why BTH occurs, as a bypass mechanism is still required to form MAC on the initiating cell, but it does offer an explanation of how an initial BTH trigger may be amplified in patients with high Type III PNH clones, commonly seen in patients receiving pegcetacoplan (75).
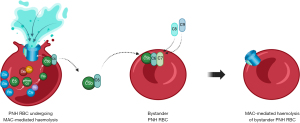
Finally, a contributing factor to BTH may be polymorphisms in complement regulators. Given the interplay between FI and the RCA family, it is likely that reduced activity of any of these proteins could contribute to increased cell surface C3, C4 and the C3 and C5 convertases and therefore contribute to the risk of BTH.
An important consideration in BTH is the size of a patient’s PNH clone at the outset, with a higher clone representing a larger population of RBCs susceptible to lysis. By preventing extravascular hemolysis, proximal complement inhibitors cause a rise in the PNH RBC clone, and therefore the potential to cause a larger fall in the hemoglobin level during BTH events (75). Furthermore, downstream effects of complement breakthrough whilst receiving proximal complement inhibitors can be more devastating than breakthrough on terminal blockade due to the alternative pathway amplification loop; for each C5 molecule that escapes inhibition, a single MAC molecule can be produced, but for each MASP-3, FD, FB or C3 molecule that escapes inhibition, one or more C3 convertases can be generated with downstream amplification leading to multiple MAC molecules being formed (75).
Conclusions
Our understanding of this ultra-rare but devastating disease has improved dramatically, but, despite this, there remain many unanswered questions in key areas.
Although the ‘escape model’ of PNH development in the context of AA is widely accepted, we do not yet fully understand what drives the PNH cell survival advantage in preference to normal HSCs in the absence of AA, nor do we know why PNH clones expand in some patients but not others. Answering these questions could help identify patients at-risk for PNH progression and unlock the potential to make early treatment decisions or even prevent disease progression before the onset of symptoms.
Therapeutic complement inhibitor research has flourished over the past two decades and new treatments with novel mechanisms of action continue to be produced. With varying methods of administration, dosing frequencies and adverse effect profiles, what is best for one patient may not suit another. With C5 inhibitors there is no way to predict which patients are most likely to experience extravascular hemolysis and require ongoing transfusion support. For all complement inhibitors, BTH is a real concern and carries the risk of severe anemia and potentially fatal thrombosis. More research needs to be done to determine its pathophysiology with an aim to identify which patients are at highest risk and how it can be prevented. Treatment responses can, in part, be affected by patient-to-patient variations in complement regulators; FH has already been identified as a potential therapeutic target (77,78) and further research into other complement regulators could yet yield further advances.
Only with further high-quality research can evidence-based individualised treatment approaches be offered to patients to limit the impact of this disease.
Acknowledgments
Funding: None.
Footnote
Peer Review File: Available at https://aob.amegroups.com/article/view/10.21037/aob-24-25/prf
Conflicts of Interest: All authors have completed the ICMJE uniform disclosure form (available at https://aob.amegroups.com/article/view/10.21037/aob-24-25/coif). M.H. reports research funding from Sobi and support with congress travel from Alexion. D.J.N. reports research funding from Sobi, Apellis and Q32bio. R.J.K. reports research funding from Novartis and Sobi; reports consultancy for Florio, Sobi, Alexion, Otsuka, and Novartis; reports speaker fees from Alexion rare disease, AstraZeneca, Novartis, Sobi, Otsuka, and Astellas; and serving as advisory board member for Alexion rare disease, AstraZeneca, Sobi, Novartis, Roche, and Jazz. D.J.N. and R.J.K. are both supported in part by the National Institute for Health and Care Research (NIHR) Leeds Biomedical Research Centre (BRC) (NIHR203331). The views expressed are those of the author(s) and not necessarily those of the NHS, the NIHR or the Department of Health and Social Care. M.G. reports consultancy for BioCryst, Regeneron, and Swedish Orphan Biovitrum AB; reports speaker fees from Alexion, AstraZeneca, Novartis, and Swedish Orphan Biovitrum AB; and serving as scientific advisory board member for Alexion, AstraZeneca, BioCryst, and Novartis. C.Q.S. is a named inventor of patent applications that describe the use of complement inhibitors for therapeutic applications. C.Q.S. has received research funding from pharmaceutical companies, honoraria for speaking at symposia organized by Alexion Pharmaceuticals, Sobi, and Sanofi; and serving on an advisory committee for Sobi. The authors have no other conflicts of interest to declare.
Ethical Statement: The authors are accountable for all aspects of the work in ensuring that questions related to the accuracy or integrity of any part of the work are appropriately investigated and resolved.
Open Access Statement: This is an Open Access article distributed in accordance with the Creative Commons Attribution-NonCommercial-NoDerivs 4.0 International License (CC BY-NC-ND 4.0), which permits the non-commercial replication and distribution of the article with the strict proviso that no changes or edits are made and the original work is properly cited (including links to both the formal publication through the relevant DOI and the license). See: https://creativecommons.org/licenses/by-nc-nd/4.0/.
References
- Hillmen P, Lewis SM, Bessler M, et al. Natural history of paroxysmal nocturnal hemoglobinuria. N Engl J Med 1995;333:1253-8. [Crossref] [PubMed]
- Gavriilaki E, de Latour RP, Risitano AM. Advancing therapeutic complement inhibition in hematologic diseases: PNH and beyond. Blood 2022;139:3571-82. [Crossref] [PubMed]
- Walport MJ. Complement. First of two parts. N Engl J Med 2001;344:1058-66. [Crossref] [PubMed]
- Varela JC, Tomlinson S. Complement: an overview for the clinician. Hematol Oncol Clin North Am 2015;29:409-27. [Crossref] [PubMed]
- Meyer S, Leusen JH, Boross P. Regulation of complement and modulation of its activity in monoclonal antibody therapy of cancer. MAbs 2014;6:1133-44. [Crossref] [PubMed]
- Wagner E, Frank MM. Therapeutic potential of complement modulation. Nat Rev Drug Discov 2010;9:43-56. [Crossref] [PubMed]
- Hayashi M, Machida T, Ishida Y, et al. Cutting Edge: Role of MASP-3 in the Physiological Activation of Factor D of the Alternative Complement Pathway. J Immunol 2019;203:1411-6. [Crossref] [PubMed]
- Mannes M, Dopler A, Zolk O, et al. Complement inhibition at the level of C3 or C5: mechanistic reasons for ongoing terminal pathway activity. Blood 2021;137:443-55. [Crossref] [PubMed]
- Jore MM, Johnson S, Sheppard D, et al. Structural basis for therapeutic inhibition of complement C5. Nat Struct Mol Biol 2016;23:378-86. [Crossref] [PubMed]
- Berends ET, Gorham RD Jr, Ruyken M, et al. Molecular insights into the surface-specific arrangement of complement C5 convertase enzymes. BMC Biol 2015;13:93. [Crossref] [PubMed]
- Harder MJ, Kuhn N, Schrezenmeier H, et al. Incomplete inhibition by eculizumab: mechanistic evidence for residual C5 activity during strong complement activation. Blood 2017;129:970-80. [Crossref] [PubMed]
- Vogt W, Schmidt G, Von Buttlar B, et al. A new function of the activated third component of complement: binding to C5, an essential step for C5 activation. Immunology 1978;34:29-40. [PubMed]
- West EE, Woodruff T, Fremeaux-Bacchi V, et al. Complement in human disease: approved and up-and-coming therapeutics. Lancet 2024;403:392-405. [Crossref] [PubMed]
- Schmidt CQ, Lambris JD, Ricklin D. Protection of host cells by complement regulators. Immunol Rev 2016;274:152-71. [Crossref] [PubMed]
- Rodriguez de Cordoba S, Lublin DM, Rubinstein P, et al. Human genes for three complement components that regulate the activation of C3 are tightly linked. J Exp Med 1985;161:1189-95. [Crossref] [PubMed]
- Ermert D, Blom AM. C4b-binding protein: The good, the bad and the deadly. Novel functions of an old friend. Immunol Lett 2016;169:82-92. [Crossref] [PubMed]
- Morgan HP, Schmidt CQ, Guariento M, et al. Structural basis for engagement by complement factor H of C3b on a self surface. Nat Struct Mol Biol 2011;18:463-70. [Crossref] [PubMed]
- Ozen A, Comrie WA, Ardy RC, et al. CD55 Deficiency, Early-Onset Protein-Losing Enteropathy, and Thrombosis. N Engl J Med 2017;377:52-61. [Crossref] [PubMed]
- Nevo Y, Ben-Zeev B, Tabib A, et al. CD59 deficiency is associated with chronic hemolysis and childhood relapsing immune-mediated polyneuropathy. Blood 2013;121:129-35. [Crossref] [PubMed]
- Kinoshita T. Glycosylphosphatidylinositol (GPI) Anchors: Biochemistry and Cell Biology: Introduction to a Thematic Review Series. J Lipid Res 2016;57:4-5. [Crossref] [PubMed]
- Rosse WF, Adams JP, Thorpe AM. The population of cells in paroxysmal nocturnal haemoglobinuria of intermediate sensitivity to complement lysis: significance and mechanism of increased immune lysis. Br J Haematol 1974;28:181-90. [Crossref] [PubMed]
- Yamakawa PE, Fonseca AR, Guerreiro da Silva IDC, et al. Biochemical phenotyping of paroxysmal nocturnal hemoglobinuria reveals solute carriers and β-oxidation deficiencies. PLoS One 2023;18:e0289285. [Crossref] [PubMed]
- Guarnera L, Visconte V. The metabolic fuel of paroxysmal nocturnal haemoglobinuria. Br J Haematol 2024;204:2162-4. [Crossref] [PubMed]
- Chen Y, Liu H, Wang C, et al. The histone demethylase JMJD1C regulates CPS1 expression and promotes the proliferation of paroxysmal nocturnal haemoglobinuria clones through cell metabolic reprogramming. Br J Haematol 2024;204:2468-79. [Crossref] [PubMed]
- Liu SS, Liu YS, Guo XY, et al. A knockout cell library of GPI biosynthetic genes for functional studies of GPI-anchored proteins. Commun Biol 2021;4:777. [Crossref] [PubMed]
- Vidugiriene J, Menon AK. Early lipid intermediates in glycosyl-phosphatidylinositol anchor assembly are synthesized in the ER and located in the cytoplasmic leaflet of the ER membrane bilayer. J Cell Biol 1993;121:987-96. [Crossref] [PubMed]
- Watanabe R, Inoue N, Westfall B, et al. The first step of glycosylphosphatidylinositol biosynthesis is mediated by a complex of PIG-A, PIG-H, PIG-C and GPI1. EMBO J 1998;17:877-85. [Crossref] [PubMed]
- Rosti V. The molecular basis of paroxysmal nocturnal hemoglobinuria. Haematologica 2000;85:82-7. [PubMed]
- Bessler M, Mason PJ, Hillmen P, et al. Paroxysmal nocturnal haemoglobinuria (PNH) is caused by somatic mutations in the PIG-A gene. EMBO J 1994;13:110-7. [Crossref] [PubMed]
- Mortazavi Y, Merk B, McIntosh J, et al. The spectrum of PIG-A gene mutations in aplastic anemia/paroxysmal nocturnal hemoglobinuria (AA/PNH): a high incidence of multiple mutations and evidence of a mutational hot spot. Blood 2003;101:2833-41. [Crossref] [PubMed]
- Pike A, Richards SJ, McKinley CE, et al. Paroxysmal Nocturnal Haemoglobinuria (PNH) Arising from Non-Canonical Mutations. Blood 2023;142:4097. [Crossref]
- Hu R, Mukhina GL, Piantadosi S, et al. PIG-A mutations in normal hematopoiesis. Blood 2005;105:3848-54. [Crossref] [PubMed]
- Araten DJ, Nafa K, Pakdeesuwan K, et al. Clonal populations of hematopoietic cells with paroxysmal nocturnal hemoglobinuria genotype and phenotype are present in normal individuals. Proc Natl Acad Sci U S A 1999;96:5209-14. [Crossref] [PubMed]
- Araten DJ, Bessler M, McKenzie S, et al. Dynamics of hematopoiesis in paroxysmal nocturnal hemoglobinuria (PNH): no evidence for intrinsic growth advantage of PNH clones. Leukemia 2002;16:2243-8. [Crossref] [PubMed]
- Young NS, Maciejewski JP, Sloand E, et al. The relationship of aplastic anemia and PNH. Int J Hematol 2002;76:168-72. [Crossref] [PubMed]
- Colden MA, Kumar S, Munkhbileg B, et al. Insights Into the Emergence of Paroxysmal Nocturnal Hemoglobinuria. Front Immunol 2021;12:830172. [Crossref] [PubMed]
- Luzzatto L, Risitano AM. Advances in understanding the pathogenesis of acquired aplastic anaemia. Br J Haematol 2018;182:758-76. [Crossref] [PubMed]
- Murakami Y, Inoue N, Shichishima T, et al. Deregulated expression of HMGA2 is implicated in clonal expansion of PIGA deficient cells in paroxysmal nocturnal haemoglobinuria. Br J Haematol 2012;156:383-7. [Crossref] [PubMed]
- Bravo-Perez C, Guarnera L, Williams ND, et al. Paroxysmal Nocturnal Hemoglobinuria: Biology and Treatment. Medicina (Kaunas) 2023;59:1612. [Crossref] [PubMed]
- Kelly RJ, Holt M, Vidler J, et al. Treatment outcomes of complement protein C5 inhibition in 509 UK patients with paroxysmal nocturnal hemoglobinuria. Blood 2024;143:1157-66. [Crossref] [PubMed]
- Peng G, Yang W, Jing L, et al. Iron Deficiency in Patients with Paroxysmal Nocturnal Hemoglobinuria: A Cross-Sectional Survey from a Single Institution in China. Med Sci Monit 2018;24:7256-63. [Crossref] [PubMed]
- Schechter AN, Gladwin MT. Hemoglobin and the paracrine and endocrine functions of nitric oxide. N Engl J Med 2003;348:1483-5. [Crossref] [PubMed]
- Ram R, Adiraju KP, Gudithi S, et al. Renal Manifestations in Paroxysmal Nocturnal Hemoglobinuria. Indian J Nephrol 2017;27:289-93. [Crossref] [PubMed]
- Hill A, Kelly RJ, Hillmen P. Thrombosis in paroxysmal nocturnal hemoglobinuria. Blood 2013;121:4985-96; quiz 5105. [Crossref] [PubMed]
- Catani MV, Bernassola F, Rossi A, et al. Inhibition of clotting factor XIII activity by nitric oxide. Biochem Biophys Res Commun 1998;249:275-8. [Crossref] [PubMed]
- Simionatto CS, Cabal R, Jones RL, et al. Thrombophlebitis and disturbed hemostasis following administration of intravenous hematin in normal volunteers. Am J Med 1988;85:538-40. [Crossref] [PubMed]
- Hoekstra J, Leebeek FW, Plessier A, et al. Paroxysmal nocturnal hemoglobinuria in Budd-Chiari syndrome: findings from a cohort study. J Hepatol 2009;51:696-706. [Crossref] [PubMed]
- Höchsmann B, Peffault de Latour R, Hill A, et al. Risk factors for thromboembolic events in patients with paroxysmal nocturnal hemoglobinuria (PNH): a nested case-control study in the International PNH Registry. Ann Hematol 2023;102:2979-88. [Crossref] [PubMed]
- Griffin M, Hillmen P, Munir T, et al. Significant hemolysis is not required for thrombosis in paroxysmal nocturnal hemoglobinuria. Haematologica 2019;104:e94-6. [Crossref] [PubMed]
- Hillmen P, Hall C, Marsh JC, et al. Effect of eculizumab on hemolysis and transfusion requirements in patients with paroxysmal nocturnal hemoglobinuria. N Engl J Med 2004;350:552-9. [Crossref] [PubMed]
- Nishimura J, Yamamoto M, Hayashi S, et al. Genetic variants in C5 and poor response to eculizumab. N Engl J Med 2014;370:632-9. [Crossref] [PubMed]
- Lee H, Park J, Jang H, et al. Population pharmacokinetic, pharmacodynamic and efficacy modeling of SB12 (proposed eculizumab biosimilar) and reference eculizumab. Eur J Clin Pharmacol 2024;80:1325-38. [Crossref] [PubMed]
- Vu T, Wiendl H, Katsuno M, et al. Ravulizumab in Myasthenia Gravis: A Review of the Current Evidence. Neuropsychiatr Dis Treat 2023;19:2639-55. [Crossref] [PubMed]
- Lee JW, Sicre de Fontbrune F, Wong Lee Lee L, et al. Ravulizumab (ALXN1210) vs eculizumab in adult patients with PNH naive to complement inhibitors: the 301 study. Blood 2019;133:530-9. [Crossref] [PubMed]
- Fukuzawa T, Sampei Z, Haraya K, et al. Long lasting neutralization of C5 by SKY59, a novel recycling antibody, is a potential therapy for complement-mediated diseases. Sci Rep 2017;7:1080. [Crossref] [PubMed]
- Röth A, He G, Tong H, et al. Phase 3 randomized COMMODORE 2 trial: Crovalimab versus eculizumab in patients with paroxysmal nocturnal hemoglobinuria naive to complement inhibition. Am J Hematol 2024;99:1768-77. [Crossref] [PubMed]
- Kelly RJ, Houghton N, Munir T, et al. 52-Week Open-Label Extension Data from a Phase 2 Study Evaluating the Safety and Efficacy of Pozelimab and Cemdisiran Combination Therapy in Patients with Paroxysmal Nocturnal Hemoglobinuria Who Switched from Eculizumab. Blood 2023;142:2716. [Crossref]
- Apellis Pharmaceuticals Inc. EMPAVELITM (pegcetacoplan) injection, for subcutaneous use: US prescribing information [Internet]. 2021 [cited 2024 Jul 23]. Available online: https://pi.apellis.com/files/PI_Empaveli.pdf
- NICE guideline. Pegcetacoplan for treating paroxysmal nocturnal haemoglobinuria [Internet]. 2022 Mar [cited 2024 Jul 23]. Available online: https://www.nice.org.uk/guidance/ta778
- Peffault de Latour R, Röth A, Kulasekararaj AG, et al. Oral Iptacopan Monotherapy in Paroxysmal Nocturnal Hemoglobinuria. N Engl J Med 2024;390:994-1008. [Crossref] [PubMed]
- Risitano AM, Kulasekararaj AG, Lee JW, et al. Danicopan: an oral complement factor D inhibitor for paroxysmal nocturnal hemoglobinuria. Haematologica 2021;106:3188-97. [Crossref] [PubMed]
- Karnabeda O, Moskalenko V, Lysak Z, et al. OMS906, a Novel Alternative Pathway MASP-3 Inhibitor, Normalizes Hemoglobin Levels and Increases Clone Size in Treatment-Naïve PNH Patients. Blood 2023;142:573. [Crossref]
- Rondelli T, Risitano AM, Peffault de Latour R, et al. Polymorphism of the complement receptor 1 gene correlates with the hematologic response to eculizumab in patients with paroxysmal nocturnal hemoglobinuria. Haematologica 2014;99:262-6. [Crossref] [PubMed]
- Prata PH, Galimard JE, Sicre de Fontbrune F, et al. Rare germline complement factor H variants in patients with paroxysmal nocturnal hemoglobinuria. Blood 2023;141:1812-6. [Crossref] [PubMed]
- Zhang L, Chen JY, Kerr C, et al. Reduced red blood cell surface level of Factor H as a mechanism underlying paroxysmal nocturnal hemoglobinuria. Leukemia 2021;35:1176-87. [Crossref] [PubMed]
- de Jong S, Volokhina EB, de Breuk A, et al. Effect of rare coding variants in the CFI gene on Factor I expression levels. Hum Mol Genet 2020;29:2313-24. [Crossref] [PubMed]
- McNamara LA, Topaz N, Wang X, et al. High Risk for Invasive Meningococcal Disease Among Patients Receiving Eculizumab (Soliris) Despite Receipt of Meningococcal Vaccine. MMWR Morb Mortal Wkly Rep 2017;66:734-7. [Crossref] [PubMed]
- Girmenia C, Barcellini W, Bianchi P, et al. Management of infection in PNH patients treated with eculizumab or other complement inhibitors: Unmet clinical needs. Blood Rev 2023;58:101013. [Crossref] [PubMed]
- Singh P, Kemper C. Complement, complosome, and complotype: A perspective. Eur J Immunol 2023;53:e2250042. [Crossref] [PubMed]
- Ram S, Lewis LA, Rice PA. Infections of people with complement deficiencies and patients who have undergone splenectomy. Clin Microbiol Rev 2010;23:740-80. [Crossref] [PubMed]
- Schaap CCM, Grotens A, de Haan AFJ, et al. Infections during eculizumab therapy in a Dutch population of patients with paroxysmal nocturnal haemoglobinuria. Clin Microbiol Infect 2021;27:1534-6. [Crossref] [PubMed]
- Risitano AM, Notaro R, Marando L, et al. Complement fraction 3 binding on erythrocytes as additional mechanism of disease in paroxysmal nocturnal hemoglobinuria patients treated by eculizumab. Blood 2009;113:4094-100. [Crossref] [PubMed]
- McKinley CE, Richards SJ, Munir T, et al. Extravascular Hemolysis Due to C3-Loading in Patients with PNH Treated with Eculizumab: Defining the Clinical Syndrome. Blood 2017;130:3471.
- Brodsky RA, Peffault de Latour R, Rottinghaus ST, et al. Characterization of breakthrough hemolysis events observed in the phase 3 randomized studies of ravulizumab versus eculizumab in adults with paroxysmal nocturnal hemoglobinuria. Haematologica 2021;106:230-7. [Crossref] [PubMed]
- Notaro R, Luzzatto L. Breakthrough Hemolysis in PNH with Proximal or Terminal Complement Inhibition. N Engl J Med 2022;387:160-6. [Crossref] [PubMed]
- Rosenfeld SI, Jenkins DE Jr, Leddy JP. Enhanced reactive lysis of paroxysmal nocturnal hemoglobinuria erythrocytes. Studies on C9 binding and incorporation into high molecular weight complexes. J Exp Med 1986;164:981-97. [Crossref] [PubMed]
- Zhang F, Zhang L, Yang C, et al. KP104, a Bifunctional C5 Antibody/Factor H Fusion Protein, Effectively Controls Both Intravascular and Extravascular Hemolysis: Interim Results from a Phase 2 Study in Complement Inhibitor-Naïve PNH Patients. Blood 2023;142:572. [Crossref]
- Schmidt CQ, Bai H, Lin Z, et al. Rational engineering of a minimized immune inhibitor with unique triple-targeting properties. J Immunol 2013;190:5712-21. [Crossref] [PubMed]
Cite this article as: Holt M, Newton DJ, Griffin M, Schmidt CQ, Kelly RJ. A review of the pathophysiology of paroxysmal nocturnal hemoglobinuria. Ann Blood 2024;9:34.